Definition
Saccharomyces cerevisiae (also known as “Baker’s Yeast” or “Brewer’s Yeast”) is a unicellular fungus responsible for alcohol production and bread formation. Cultured for thousands of years, S. cerevisiae undergoes fermentation to create these products. As a rapidly reproducing eukaryote, Saccharomyces cerevisiae is a widely used model organism that has allowed scientists to better understand molecular, cellular, and biochemical processes, as well as the pathology and potential treatments to common human diseases.
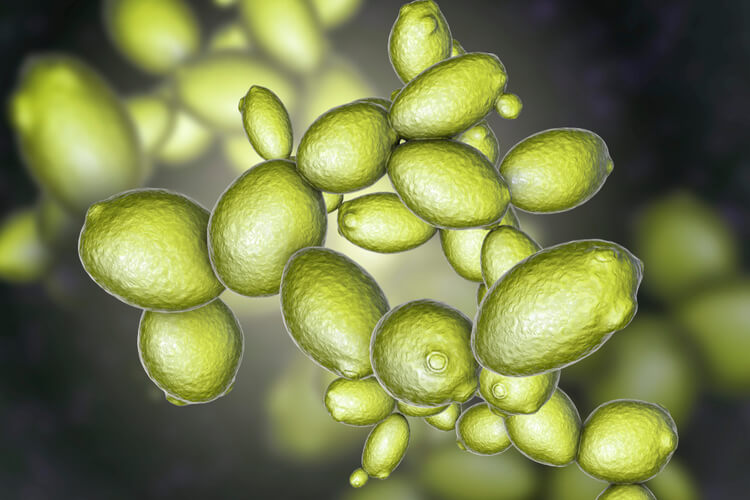
History of Saccharomyces cerevisiae
Beverage and Food Production
Saccharomyces cerevisiae (scientific name: S. cerevisiae) is a single- celled (or unicellular) fungus known commonly as yeast. It has been cultured by humans for thousands of years, as it is the organism known for producing a variety of alcoholic beverages- such as beers and wines- as well as baked goods- such as breads. Although brewers and bakers were unaware of how exactly S. cerevisiae was capable of producing these goods, scientists have extensively studied this organism since the 1800’s in an effort to better understand its processes. It is now known that S. cerevisiae contributes to alcohol and baked good productions by undergoing a biochemical process known as fermentation. Yeast fermentation became one of the first processes to be investigated in the discipline of biochemistry.
S. cerevisiae naturally grows on fruits- such as grapes and dates- as well as grains- such as wheat and barley. Its primary form of reproduction is budding, where daughter cells sprout directly off of mother cell. Some of the oldest known uses of S. cerevisiae goes back to 4,000 BCE in Ancient Egypt, where ancient Egyptians would use yeast to make bread. Following the discovery of the microscope by Anton van Leeuwenhoek in 1676, brewers were able to culture their yeast colonies by morphology (or by its physical appearance). By separating colonies by their morphologies, brewers were able to produce different tastes. By 1859, Louis Pasteur was able to generalize the process in which S. cerevisiae aided bread making, where the carbon dioxide released allowed bread to rise.
Benefits to Biological Studies
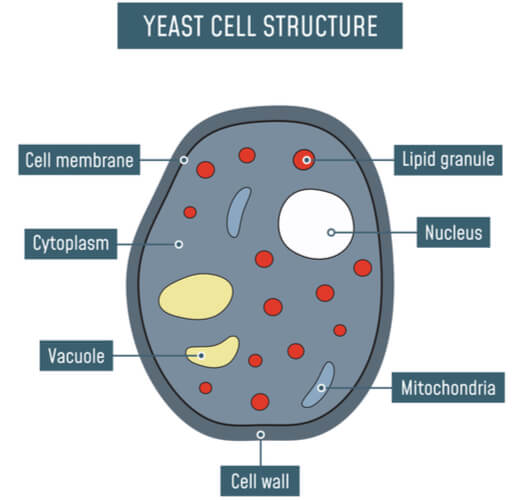
Studying of Saccharomyces cerevisiae did not end with brewing and baking. As single-celled organisms, S. cerevisiae reproduces very quickly at rates comparable to bacterial cells in good to optimal conditions. As eukaryotes, they contain several of the same cellular systems as multicellular organisms, including cell walls, cell membranes, nuclei, the endoplasmic reticulum, the Golgi apparatus, mitochondria, vacuoles, and vesicles. Combining these aspects, S. cerevisiae serves as an excellent model organism that can be easily grown and manipulated in the laboratory in order to study mechanisms and processes that better our understanding of larger concepts in biology.
Not much is known on the natural life and ecology of S. cerevisiae outside of the lab. However, research is ongoing to obtain better insights concerning this lack of information.
Saccharomyces cerevisiae in Alcohol and Bread Formation
Despite its long history in alcohol and bread production, it was not known exactly how Saccharomyces cerevisiae made these goods. By studying its biochemical processes, we now know that S. cerevisiae releases molecules of alcohol and carbon dioxide as by-products while undergoing fermentation. S. cerevisiae does not intend to create these by-products- they are simply products released as waste that are not needed for S. cerevisiae’s survival.
Fermentation is a process that many cells utilize to produce energy from sugar molecules in oxygen-lacking environments. It is a less energy-efficient alternative to cellular respiration. In order to better understand why fermentation occurs, let’s first look at the overall process of cellular respiration and its biological significance.
Cellular Respiration
Cellular respiration is the process in which sugar [in the form of glucose (C6H12O6)] is broken down to create energy in the form of adenosine triphosphate (or ATP). ATP is absolutely critical to life, as it provides the energy that cells need to complete biochemical processes throughout the body. For this reaction to occur, there must be oxygen present. Carbon dioxide (CO2) and water (H2O) are also released by the end of the reaction.
The equation used to represent the chemical breakdown of cellular respiration is as follows:
Glucose + 6 Oxygen —> 6 Water + 6 Carbon dioxide + 29 ATP
or
C6H12O6+ 6 O2 —-> 6 H2O + 6 CO2+ 29 ATP
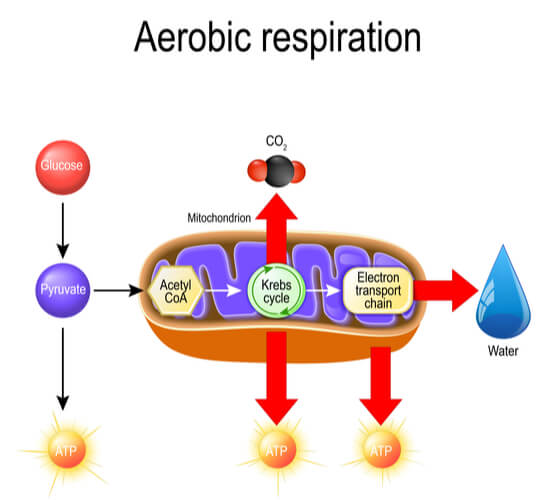
The full process of cellular respiration is made up of four steps: glycolysis, pyruvate processing, the tricarboxylic acid cycle (or TCA Cycle, also known as the Krebs Cycle and Citric Acid Cycle), and the electron transport chain (or ETC). Cellular respiration is evolutionarily important because the full process produces 29 ATP molecules from a single glucose molecule. Most of these ATP molecules are produced in the last step of the ETC. However, some ATP is also produced in the steps leading up to the ETC- most notably in glycolysis.
Glycolysis
Glycolysis (glyco= sugar, lysis= breakdown) is a 10-step process in which one glucose molecule (which contains six carbons) is broken down into two pyruvate molecules (each containing three carbons). It takes two ATP molecules to jumpstart glycolysis, where four molecules are produced by the end. As a result, only a net of two ATP molecules is created during glycolysis.
Another critical molecule in glycolysis is nicotinamide adenine dinucleotide (or NADH). As a redox- reaction, it is important to note that NAD+ is the oxidized form of NADH, and NADH is the reduced form of NAD+. Oxidation is the loss of an electron, while reduction is the gain of an electron (pneumonic: OIL RIG= Oxidation Is Loss, Reduction Is Gain!), where the addition of a hydrogen is commonly the source of the new electron. Therefore, NADH is oxidized to become NAD+, while NAD+ is reduced to become NADH. During the process of glycolysis specifically, two molecules of NAD+ is reduced to create two molecules of NADH.
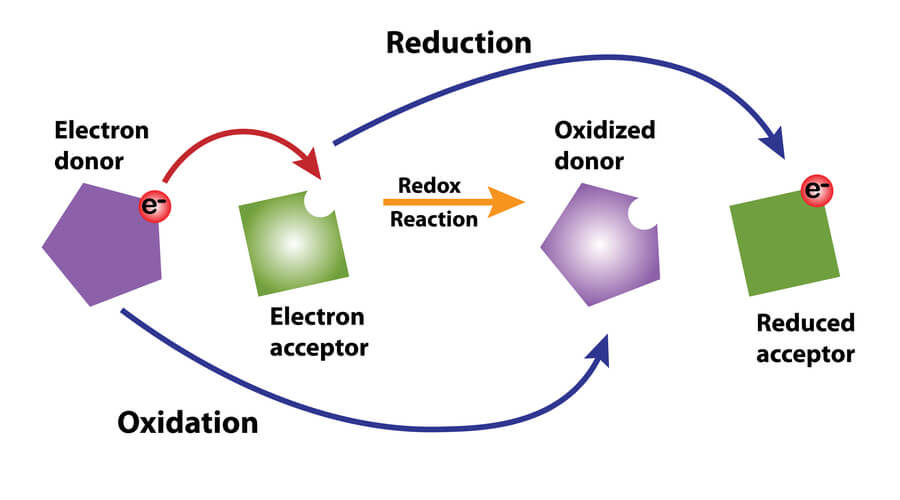
Therefore, the overall equation of glycolysis is as follows:
Glucose + 2 NAD++ 2 ADP + 2 Pi —> 2 Pyruvate + 2 NADH + 2 H++ 2 ATP + 2 H2O
As shown by the overall cellular respiration reaction from the previous section, oxygen (O2) is critical for the process to occur. While oxygen is not used during glycolysis, it must to be present in order for the cell to continue past glycolysis and into the next steps of cellular respiration. If oxygen is not present, then the cell will instead undergo fermentation.
Fermentation
Fermentation is the process in which glucose is broken down in an anaerobic (or oxygen- lacking) environment. There are many different types of fermentation, with two of the most well-known being lactic acid fermentation and ethanol fermentation. While muscle cells can undergo lactic acid fermentation, yeast cells specifically undergo ethanol fermentation. Therefore, we will only focus on ethanol fermentation for this article.
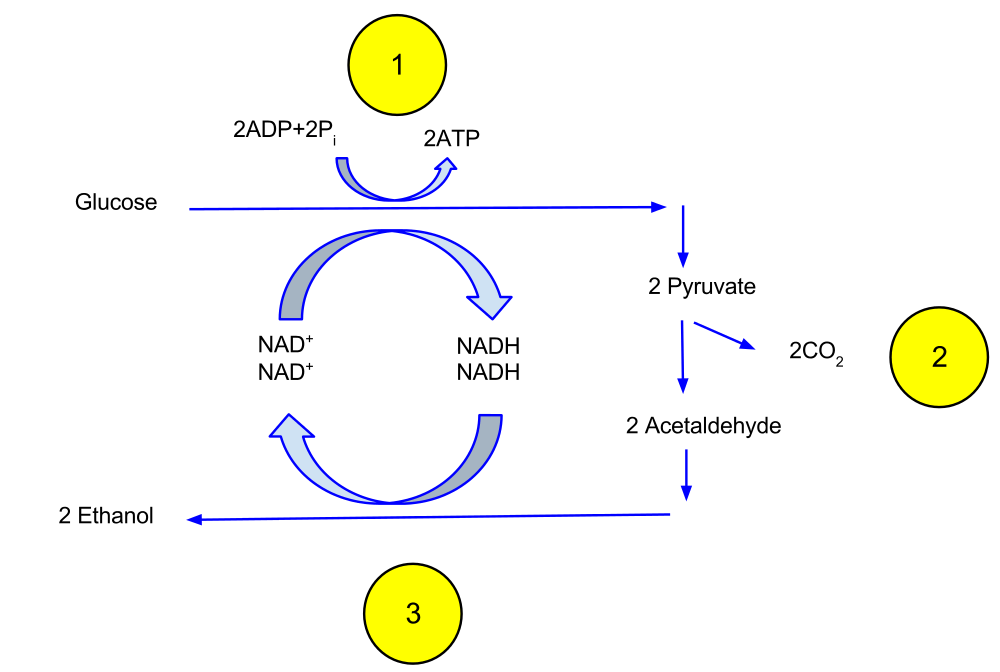
Similar to the process of cellular respiration, Saccharomyces cerevisiae undergoes glycolysis to produce two molecules of pyruvate, as previously described. Thus, it also produces two molecules of ATP and 2 molecules of NADH. Following glycolysis however, S. cerevisiae converts the two pyruvate molecules (each containing 3 carbons, for a total of six carbons) into two acetaldehyde molecules (each containing two carbons, for a total of four carbons). The two missing carbons are released from the yeast cells as waste by- products in the form of carbon dioxide (CO2). This is the gas that allows bread to rise during bread making.
The NADH molecules that were produced during glycolysis then interact with the acetaldehyde molecules. NADH passes acetaldehyde an electron so that NADH is oxidized back to NAD+. Now, the NAD+ can be reused for glycolysis. When acetaldehyde receives this electron, it is reduced to ethanol. The ethanol is released from the yeast cells as waste by- products. As ethanol is an alcoholic molecule, this is the product responsible for alcoholic beverage production.
The Process of Beer Brewing
The process of beer brewing involves multiple steps over the course of a few to several weeks.
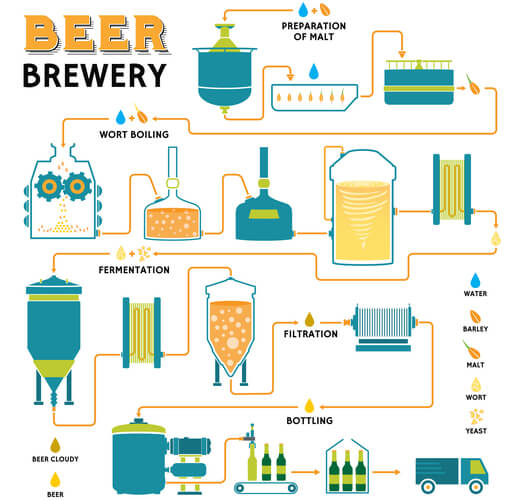
“Malting” is the first step of brewing, where carbohydrates from grains (such as barley) germinate. During germination, degradation enzymes- such as α-amylase and maltase- are turned on. “Mashing” is the second step, where the grains are crushed to release metabolic enzymes. Water is then added to create a “wort” mixture. The wort is incubated at temperatures that optimize the enzymes’ catalytic activity. This allows the carbohydrates to be broken down and ready to enter glycolysis. Anything undigested is removed by filtration.
The next step is to boil the wort and add flavoring ingredients, such as hops (which are plants that add bitterness and scents to the beer). Once this mixture cools, a live yeast culture is added. Oxygen is still left in the mixture to allow the culture to grow over a short period of time. Once a large enough culture is grown, oxygen is removed from the system, and the yeast ferments the carbohydrate metabolites. As ethanol is released, beer is produced.
In large scale breweries, the beer is filtered, and additional CO2 is added before the beverage is bottled. However, in small scale breweries, additional yeast and sugar is added to create a second fermentation step during bottling. The additional CO2 results in carbonation, allowing the drinks to become “fizzy”.
Though beer is primarily thought of when discussing Saccharomyces cerevisiae, multiple alcoholic beverages are created using this yeast species. By using various strains, cultures, raw carbohydrates, flavors, and methodologies, it is possible to create wine, cider, mead, whisky, cognac, rum, vodka, grappa, tsiporo, and gin from S. cerevisiae as well.
Saccharomyces cerevisiae as a Model Organism
The uses for Saccharomyces cerevisiae go far beyond brewing and baking and have allowed scientists to make thousands of discoveries that better our understandings in genetics, molecular biology, cellular biology, biochemistry, and much more. As single-celled organisms, S. cerevisiae is able to quickly reproduce and thrive in laboratory settings. The average cell cycle for a single yeast cell in optimal conditions is around 90 minutes, meaning a sufficient amount of yeast can be grown within hours. Additionally, yeast can be grown in acidic, high sugar conditions. These conditions prevent bacterial growth, thus avoiding contamination and conflicting results.
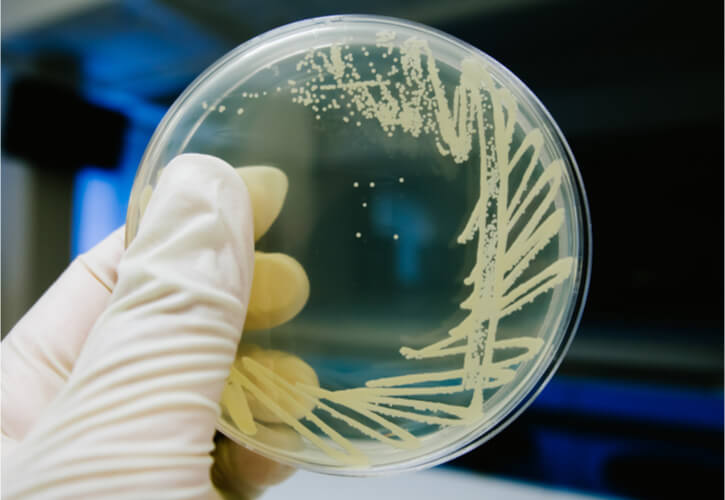
As eukaryotes, S. cerevisiae contains molecular, cellular, and biochemical similarities to more complex eukaryotic organisms, including humans. Therefore, S. cerevisiae offers itself as a useful model organism. Specifically, experiments can be undertaken to better understand these processes and their applications in more complex organisms, especially when considering diseases and treatments. Being the first eukaryotic organism to have its entire genome sequence, it was quickly discovered that S. cerevisiae contained at least 31% of functioning genes with homologs (or equivalent genes) in humans. Additionally, at least 20% of genes that play important roles in human disease also have homologs in yeast cells. Therefore, by studying yeast, scientists have made advancements that better our understanding in concepts such as cell cycle regulation and division, DNA repair, and much more.
Cell Cycle Research and Cancer
Leland Hartwell, a biologist, used Saccharomyces cerevisiae as a model to better understand the eukaryotic cell cycle, as well as mutations in the cell cycle that lead to cancer. Specifically, he completed this by studying mutated genes in S. cerevisiae. In doing so, he determined the role each gene played in the cell cycle when it was no longer functional. By identifying over 100 genes, Hartwell ultimately discovered that genes that should suppress cancer no longer worked when mutated, and genes that push the cell cycle forward cannot be turned off when mutated. As a result, the cell loses its ability to shut down and continues to divide despite signals to stop. These now cancerous cells continue to divide until they ultimately form tumors. By identifying these genes, additional scientists were able to identify key proteins that also assist cell cycle progression.
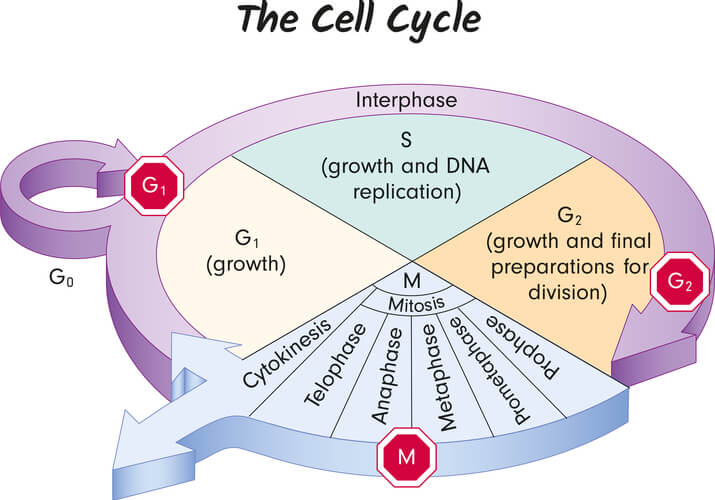
In addition to studying mutated genes in the cell cycle, Hartwell also determined multiple “checkpoint” genes, which are genes that supervise cell cycle status. At each checkpoint, various proteins ensure specific requirements are met. If the requirements are met, the checkpoint genes allow the cell to progress through the cell cycle. If there are issues, such as a loss in DNA integrity or insufficient amounts of specific proteins, the checkpoint genes will either halt cell cycle progression or induce apoptosis (or programmed cell death). Hartwell identified that mutations in these checkpoint genes play a large role in cancer formation. This is because mutated checkpoint genes cannot properly perform their roles, and can give off signals for the cell to continue dividing even when there are problems that should instead result in apoptosis.
Saccharomyces cerevisiae in Neurodegenerative Research
Despite being unicellular, Saccharomyces cerevisiae contains multiple genes and proteins that are homologous to several neurodegenerative disorders in humans. These include diseases associated with protein misfolding, such as Alzheimer’s, Parkinson’s, and Huntington’s Disease. It is important to note, however, that many of these diseases mentioned are still incompletely understood. Research is ongoing to better understand why exactly accumulation of neural proteins results in these neurodegenerative diseases.
Alzheimer’s Disease
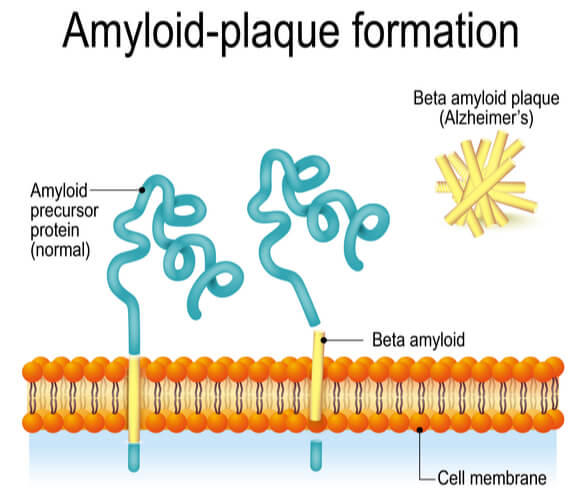
The most common neurodegenerative disease is Alzheimer’s Disease. The physical symptoms include impaired higher thinking, altered behaviors, disorientation, and memory loss. It is caused when beta amyloid peptide (Aβ) accumulates. Aβ is created when the amyloid precursor protein (APP) is cleaved by β-amyloid converting enzyme (BACE) and γ-secretase. By studying these proteins in S. cerevisiae, the secretases used in APP processing were determined. This allowed scientists to better understand the proposed process in which Alzheimer’s is formed. In addition to identifying specific secretases, the use of S. cerevisiae has allowed compounds that inhibit BACE to be tested, as well as APP fragment toxicity to be monitored in vitro.
Parkinson’s Disease
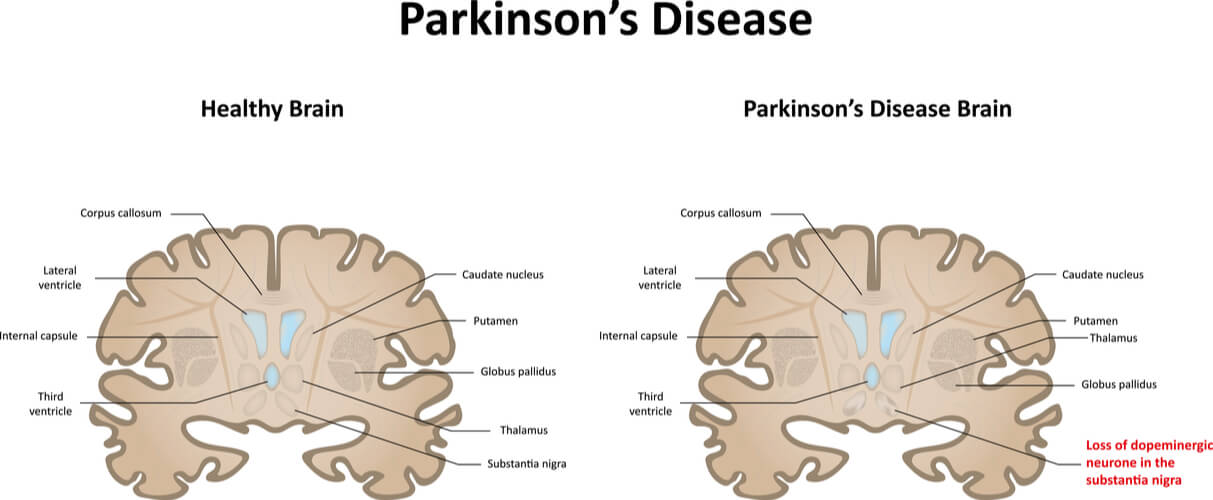
The second most common neurodegenerative disease is Parkinson’s Disease. The physical symptoms include motor instabilities- such as tremors and rigidity- which are caused by damage dopaminergic neurons in the brain. Specifically, there is a high presence of α-synculein inclusions, with α-synculein being important for vesicle transport at synapses. Studying α-synculein in S. cerevisiae allowed scientists to identify the toxicity of α-synculein in various concentrations. Additionally, it allowed scientists to identify factors that lead to increased α-synculein levels (including reactive oxygen species, endoplasmic reticulum stress, heat shock, lipid metabolism, and several genes involved with these processes, amongst others).
Huntington’s Disease
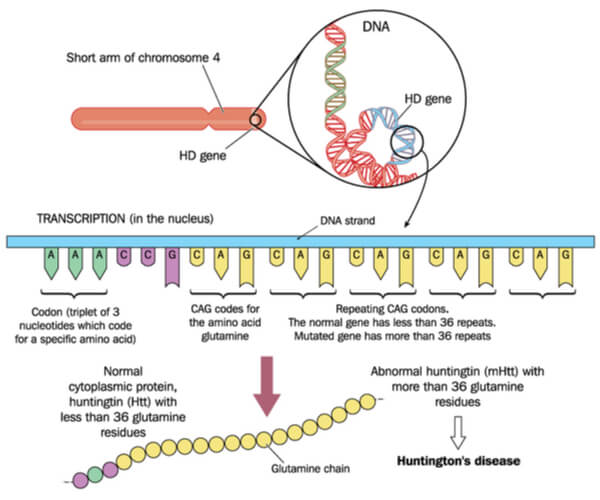
The most common polyglutamine disease is Huntington’s Disease. Physical symptoms include involuntary “jerky” movements throughout the body. This disease is caused by CAG (cytosine- adenine-guanine) repeats located on the 4p16.3 gene, which encodes for the Huntington protein. Typically, the Huntington protein is an important cell survival protein that has roles in transcription, vesicular trafficking, metabolism, and synapses. In healthy individuals, there should only be ~11-34 copies of the repeats. However, those with Huntington’s Disease instead have hundreds of CAG repeats, resulting in an expanded glutamine protein chain. Eventually, this disease results in the degeneration of the striatum of the brain (located in the forebrain). It was determined in S. cerevisiae that Huntington protein accumulation increased as the CAG codon tract expanded. However, it was also determined that toxicity of the Huntington protein only increased with the addition of specific prions (or misfolded proteins).
Conclusion
Saccharomyces cerevisiae are unicellular yeast cells that have been used for alcohol and baked good formation for thousands of years. Originally studied to better understand the process in which this occurs, the studies of this organism developed into the complex biochemical studies that have allowed researchers to better understand eukaryotic processes. S. cerevisiae offers itself as an excellent model organism, where studies continue today to better understand biological concepts and human diseases.
Quiz