AP Biology 5.2 - Meiosis and Genetic Diversity
While the last section of the AP Biology curriculum examined the processes of meiosis, this section of the curriculum looks more closely at why meiosis increases the genetic diversity in a population. We’ll start by defining genetic diversity and looking at how genetic diversity exists on the molecular level. Then, we’ll look at meiosis and the processes that distribute alleles to new daughter cells. In doing so, we’ll see how the Law of Segregation and the Law of Independent Assortment are created. The first law deals with the segregation of chromosomes, while the second law deals with how different genes are inherited independently from one another (unless they are linked on the same chromosomes). Finally, we’ll see how the process of recombination (a.k.a. crossing over) can unlink genes and further increase genetic diversity in a population.
Video Tutorial
The following video summarizes the most important aspects of this topic!
To watch more tutorial videos like this, please click here to see our full Youtube Channel!
Resources for this Standard
For Students & Teachers
- Overview & Video Tutorial (This article)
- Quick Test Prep
- Crossword Puzzle
For Teachers Only
ENDURING UNDERSTANDING
IST-1
Heritable information provides for the continuity of life.
LEARNING OBJECTIVE
IST-1.H
Explain how the process of meiosis generates genetic diversity.
ESSENTIAL KNOWLEDGE
IST-1.H.1
Separation of the homologous chromosomes in meiosis I ensures that each gamete receives a haploid (1n) set of chromosomes that comprises both maternal and paternal chromosomes.
IST-1.H.2
During meiosis I, homologous chromatids exchange genetic material via a process called “crossing over” (recombination), which increases genetic diversity among the resultant gametes.
IST-1.H.3
Sexual reproduction in eukaryotes involving gamete formation – including crossing over, the random assortment of chromosomes during meiosis, and subsequent fertilization of gametes – serves to increase variation.
EXCLUSION STATEMENT
The details of sexual reproduction cycles in various plants and animals are beyond the scope of the course and the AP Exam.
5.2 Meiosis and Genetic Diversity Overview
To understand why increasing genetic diversity through meiosis is important, we should consider the story of bananas in the 1950s. Banana farmers at the time were largely producing one variety of bananas – the Gros Michel. While it was shorter and fatter than the Cavendish variety we are used to today, it apparently had a much more appealing taste. But, since banana farmers largely relied on clones of the exact same banana plant, there was almost no genetic diversity in the banana population. So, when a banana-eating fungus swept through the Gros Michel crop, there was no genetic diversity and the entire variety was lost to the disease. Now, you can only get Cavendish bananas in the grocery store.
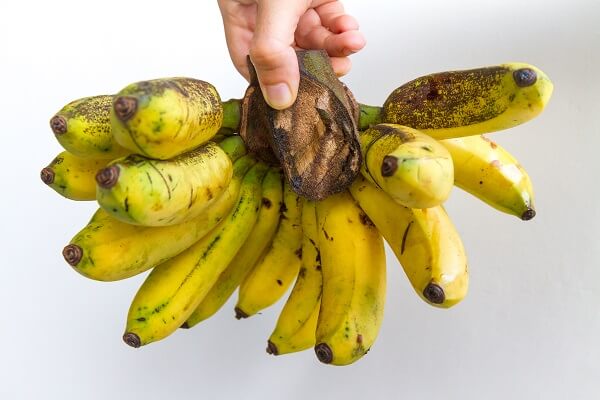
If banana farmers would have had a little foresight, they would have put Gros Michel bananas through the process of meiosis on a regular basis, they may have preserved enough genetic diversity in the crop to protect it from the banana-eating fungus. Meiosis has several mechanisms that recombine genetic variants from different lines to create unique and resistant offspring. The AP test will definitely ask a question or two on how meiosis increases genetic diversity. So, follow along as we dive into the process of meiosis and show exactly how it increases genetic variation in a population.
To understand how meiosis increases the genetic diversity of a population, we first have to understand what genetic variations are and how they can help a population survive in the face of constantly-changing environmental conditions.
Diploid organisms carry two alleles for each gene, one inherited from their mother, and the other inherited from their father. These alleles contribute to the overall phenotype (or outward appearance) of the organism. The processes of meiosis and sexual reproduction happen between each generation of organisms, mixing up the alleles that the offspring receive. This is how a red and white flower can produce pink offspring, and how these pink offspring can produce offspring that show all three color variations.
Let’s see how this genetic variation exists on the level of chromosomes. Homologous chromosomes, also known as homologs, are the chromosomes received from the maternal and paternal gametes during the process of fertilization. Each of these homologous chromosomes carries a number of genes, in the exact same location on each homolog. Keep in mind that these homologous chromosomes will never become attached at the centromere because only sister chromatids are attached at the centromere after the process of DNA replication.
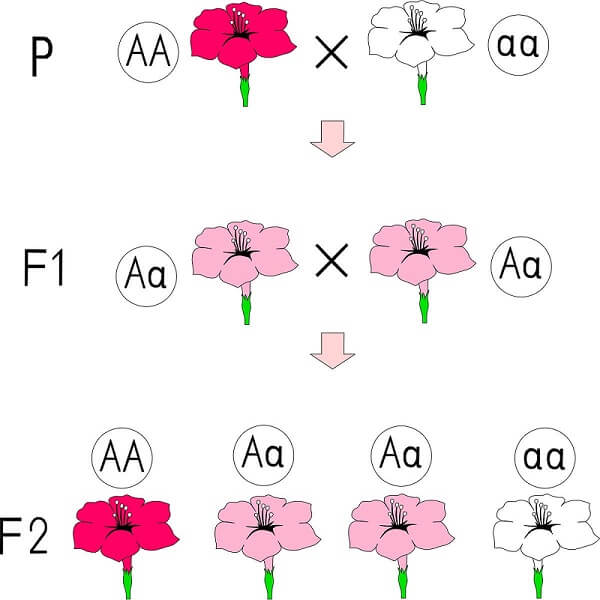
Let’s put this in the context of our theoretical flower color alleles. If these homologs carry the gene for flower color, each homolog carries an allele from a different paternal source. A red flower receives two capital “A” alleles – one from a maternal source and one from a paternal source. A white flower received two small “a” alleles from each parent – essentially meaning it has two broken copies of the gene and cannot produce pigment. A pink flower received one functional “A” allele and one non-functional “a” allele.
Keep in mind that before meiosis this cell will replicate the DNA. This will create two sister chromatids of each chromosome, effectively copying each allele so there are 4 alleles present. As we will see, these alleles will be divided up into separate gametes during meiosis and potentially mixed up by the process of crossing over.
Why is this important for a species’ survival? Well, simply because changing environmental conditions are unpredictable. Let’s say that bees like pink flowers and moths prefer white flowers. Bees may prefer pink flowers and may be better pollinators most of the time. But, if all the bees in an area get wiped out by a pesticide, then the pink flowers are doomed. If these plants only reproduced by asexual reproduction and did not use meiosis to mix up their alleles, they would all become extinct – much like the banana variety from the beginning of this video!
Now, let’s take a broad look at meiosis and the mechanisms that ensure genetic diversity is increased in future generations through the process of sexual reproduction.
Pretend that the flower-producing plant from the previous paragraph is an organism that has 2 chromosomes. Each chromosome has 2 homologs, 1 from the plant’s mother and 1 from the plant’s father. By prophase I of meiosis, each homolog has been replicated and the sister chromatids are bound together at the centromere. Here, we can see that the maternal chromosomes are purple, while the paternal chromosomes are green. By metaphase I of meiosis, the homologous chromosomes have aligned on the metaphase plate. Chromosome 1 in this example carries the gene for flower color. Remember that each homologous chromosome has been duplicated, so there are 4 alleles present – a duplicate set of maternal alleles and a duplicate set of paternal alleles.
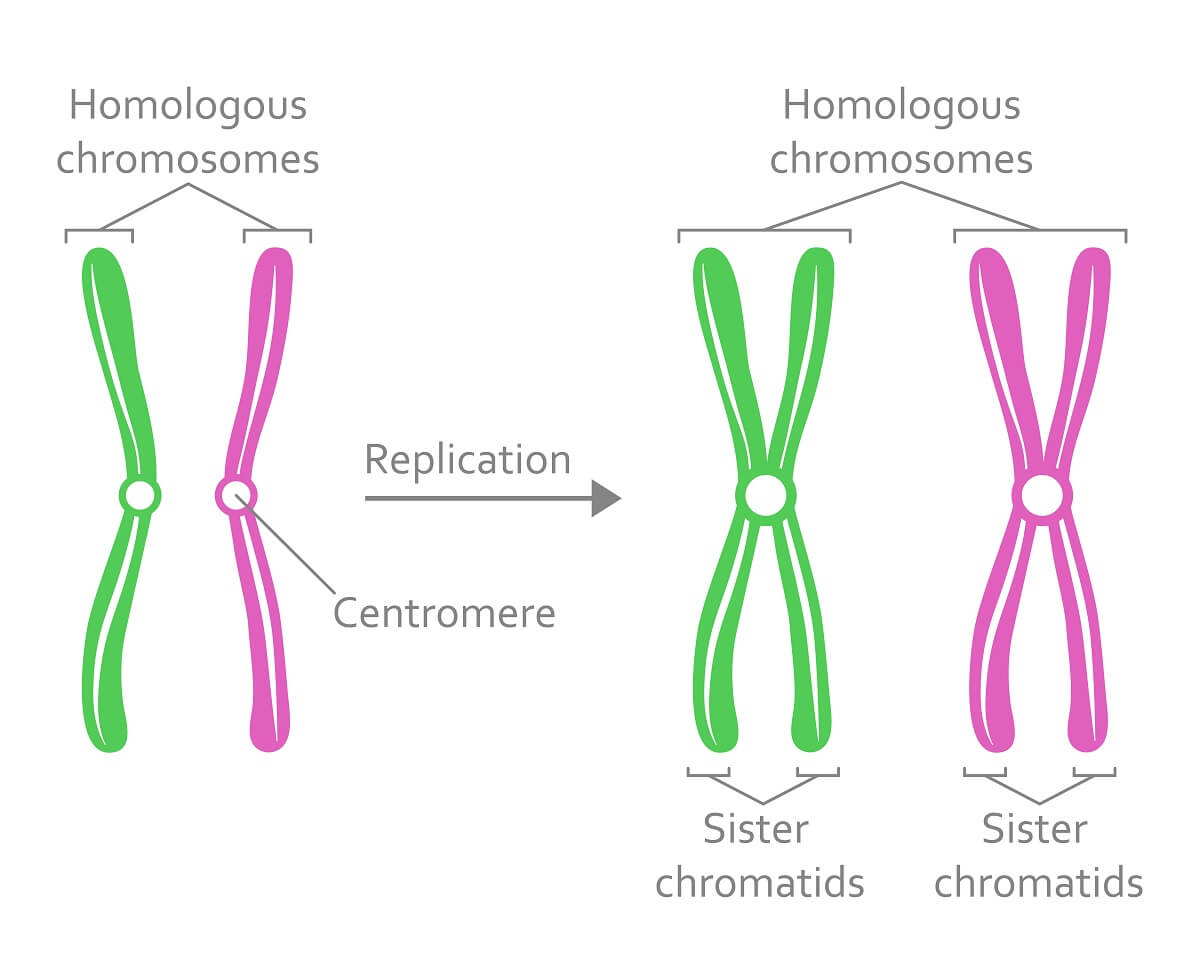
As meiosis I progresses, these maternal and paternal homologs are segregated into different cells. As meiosis II takes place, the sister chromatids in each of these cells are separated into cells of their own. Ultimately, this process distributes 1 of the 4 alleles to each of the 4 new daughter cells.
While the process of meiosis easily splits up these homologous chromosomes and the alleles they carry into individual cells, the real magic happens when you consider how all of the genes present on all of the chromosomes are randomly distributed to daughter cells. Plus, another special event called crossing over takes place during prophase I that exchanges genetic material between maternal and paternal homologs.
The process of meiosis leads to two of Mendel’s genetic laws: the law of independent assortment and the law of segregation. As a monk who enjoyed breeding and observing pea plants, Mendel had plenty of time on his hands to discover these laws simply by observing how often different traits appeared together by counting the phenotypes of the offspring.
But, with the advent of microscopes and high-throughput genetic sequencing, scientists have been able to confirm and expand on these laws. Let’s see exactly what each of these laws state. The Law of Segregation states that maternal and paternal alleles for a gene are segregated into different gametes. We saw this in the last section, as meiosis I separates homologous chromosomes, then meiosis II separates alleles located on sister chromatids.
By contrast, the Law of Independent Assortment states that different genes coding for different traits are inherited independently. For example, the gene for flower color and the gene for plant height do not affect each other. In other words, if one gamete receives the allele for purple flowers, this does not affect whether that same gamete will receive the tall allele or the short allele. This law ensures that traits are randomly assorted in new generations, creating a massive amount of genetic diversity.
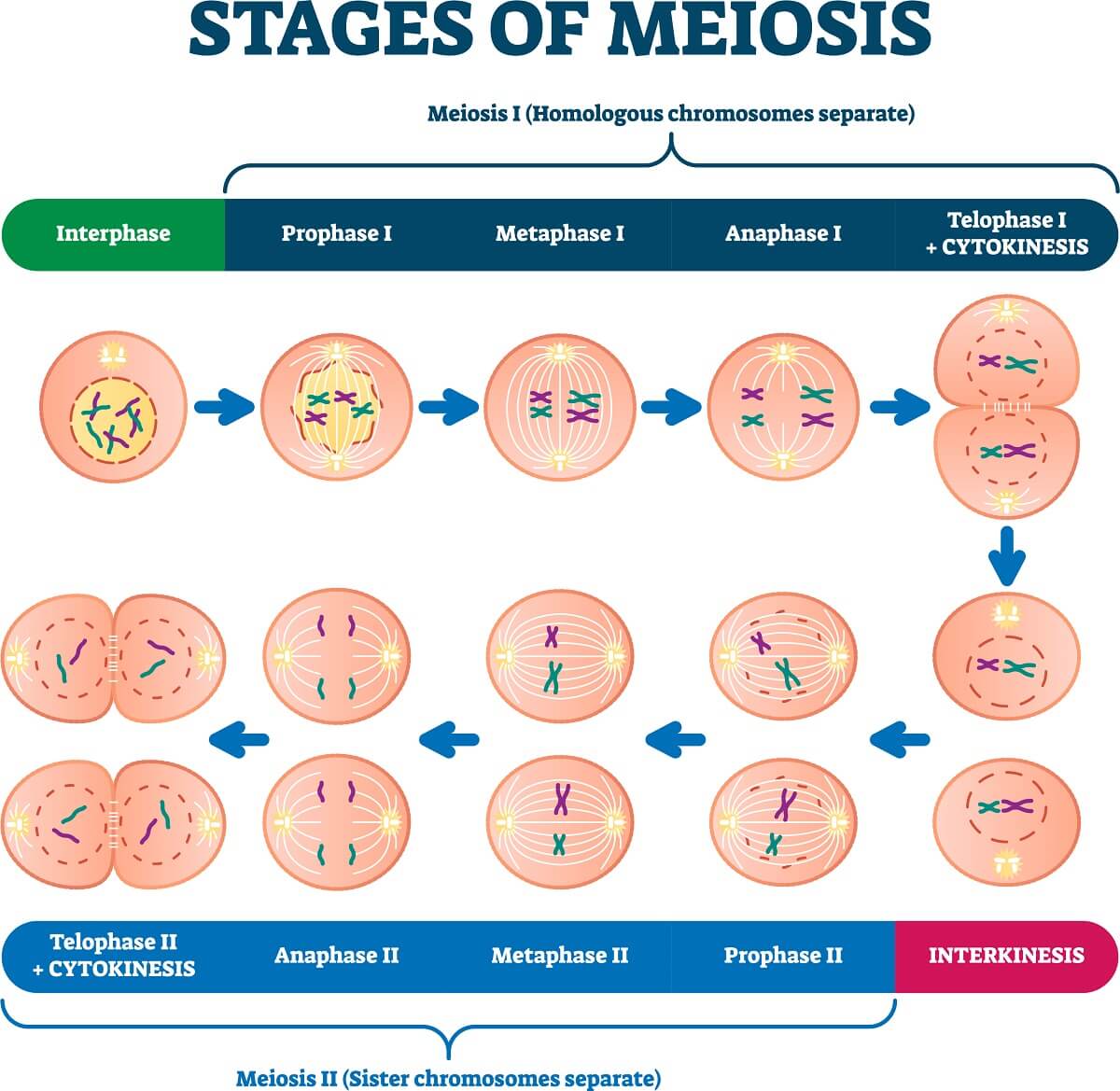
A major exception to this law is genes that are located on the same chromosome. These are known as “linked-genes” due to the fact that they are physically connected. However, meiosis has one final trick up its sleeves that can separate all but the most closely-related linked genes: crossing over.
The process of crossing over, also known as recombination, occurs during prophase I of meiosis, as the homologous chromosomes are condensing and being pulled toward the metaphase plate. Specifically, as the homologous chromosomes are condensed and collected, they are temporarily bound together by the synaptonemal complex – a series of RNAs and proteins that hold the two homologous chromosomes together.
This binding of homologous chromosomes is known as synapsis. Considering that the two non-sister chromatids that are closest to each other share the exact same sequence of genes, they have an incredibly similar structure. While the maternal and paternal homologs can carry different alleles, alleles are typically only a difference of a few nucleotides in a chromosome consisting of hundreds of millions of nucleotides.
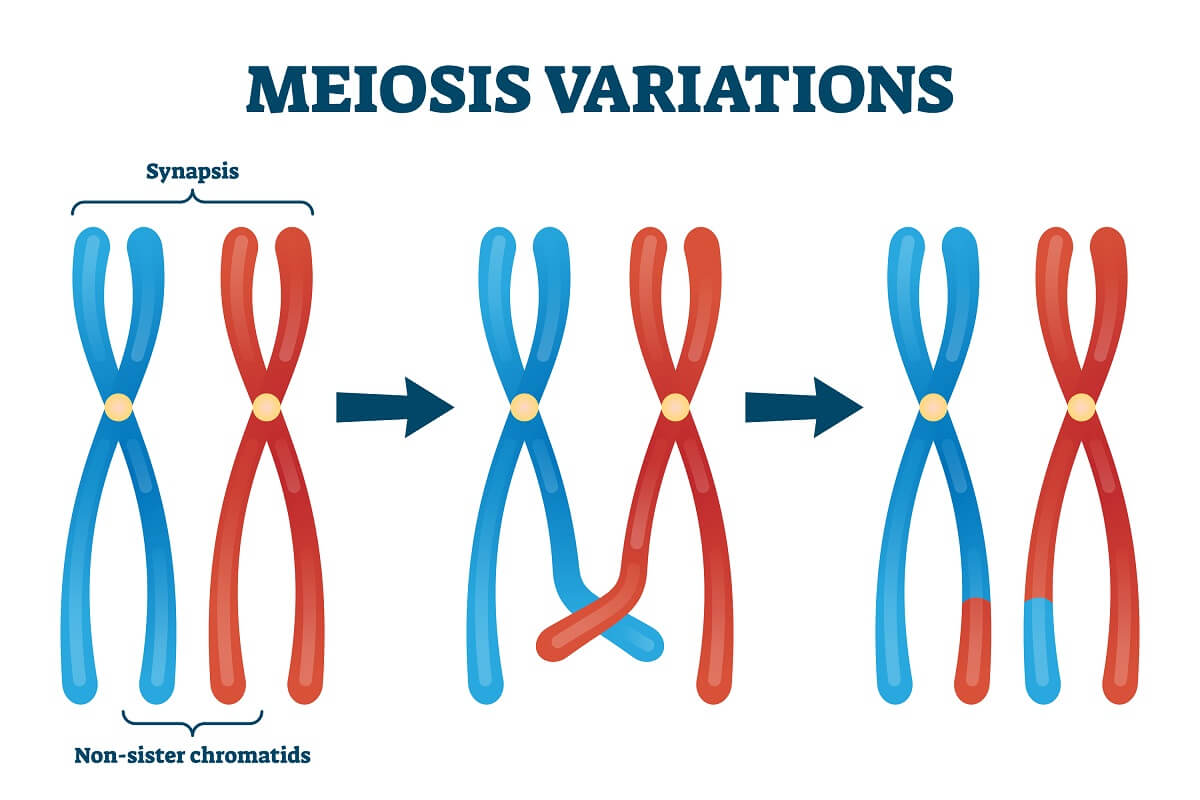
So, when these two incredibly similar non-sister chromosomes cross over one another, this is known as a chiasma. When the two non-sister chromatids are crossed over each other in this way, they have the potential to form a holiday junction. This 4 way DNA structure results when the chiasma causes breaks in similar places along each non-sister chromatid. As the DNA repair enzymes try to resolve this structure, they have a chance of mixing up the strands of DNA that have become entangled. As the synaptonemal complex breaks down and meiosis continues to completion, the recombinant chromatids are separated and end up in separate gametes.
As such, this has the effect of mixing up the alleles normally carried by maternal and paternal chromosomes. This is why no offspring look exactly like their parents or their grandparents. Even if they were to receive a full set of maternal or paternal chromosomes, the process of crossing over ensures that specific combinations of traits linked on chromosomes can be recombined between maternal and paternal homologous chromosomes!